Total Cholesterol: Diagnostic Significance and Clinical Insights
Authors: Payal Bhandari M.D., Tejal, Madison Granados
Contributors: Vivi Chador, Amer Džanković, Hailey Chin
|
Key Insights
Cholesterol, made by the liver and other organs, helps produce hormones, bile salts, vitamin D, and energy, with a small amount obtained from food. It travels through the body via protein carriers (lipoproteins), where higher protein levels mean less fat. Managing cholesterol involves a healthy diet, exercise, stress reduction, hydration, and, if necessary, medication or surgery.
What Is Cholesterol?
Cholesterol, measured in lipid panels, includes LDL, HDL, IDL, and VLDL, essential for assessing metabolism and detecting health conditions. Found in cell membranes and lipoproteins, lipids like cholesterol, triglycerides, and phospholipids are made of carbon, hydrogen, and oxygen. Triglycerides have a glycerol backbone and three fatty acids. Saturated fats have straight chains, while unsaturated fats have double bonds, making them less dense.
Figure 1: Lipid (Cholesterol) Structure
Figure 2: Lipoproteins are particles with a core of cholesterol esters and triglycerides, surrounded by free cholesterol, phospholipids, and apoproteins, which aid their formation and function1.
Figure 3: Plasma lipoproteins include chylomicrons, VLDL, IDL, LDL, HDL, and Lipoprotein A. Most are pro-atherogenic except HDL, which is anti-atherogenic. Cholesterol and triglycerides bind to apoproteins to travel in blood, as they are water-insoluble. Apoproteins aid lipoprotein formation and regulate metabolism enzymes1.
Cholesterol Synthesis
The body produces most of its cholesterol, with only a small amount coming from food like dairy, red meat, eggs, poultry, fish, shellfish, cooking oils, and nuts. About 20–25% of cholesterol is made in the liver, while 75–80% is produced in the small intestine, adrenal glands, and reproductive organs.
Regulation of Cholesterol Synthesis
Peripheral Tissue Cholesterol Synthesis
˛Peripheral tissues synthesize about 9 mg of cholesterol per kg of body weight daily, with less regulatory control than the liver, which responds to dietary, hormonal, and physiological factors. About 600–800 mg of cholesterol is transported daily from peripheral tissues to the liver for metabolism and excretion via reverse cholesterol transport (RCT). This process is regulated by6:
SREBPs: Activate cholesterol and fatty acid synthesis genes when cholesterol is low.
LDLRs: Transport LDL cholesterol into cells; high LDL levels suppress LDLR expression via SREBPs.
LXRs: Promote cholesterol removal from cells to the liver. Oxysterols activate LXRs, which pair with RXRs to regulate target genes.
De Novo Cholesterol Synthesis in the Liver
The liver regulates cholesterol synthesis for ATP production. Glucose is used for energy, stored as glycogen, or converted to FA, TAG, or VLDL. Proteins and AAs are broken down or recycled.
During fasting or exercise, the body uses FAs and proteins. Triglycerides convert to glucose or ketones via ketogenesis, activating glucagon and catecholamines. LPL breaks down glycogen and triglycerides into ketone precursors. HMG CoA synthase forms acetoacetate, converted to beta-hydroxybutyrate for energy. The liver also processes VLDL, NEFAs, AAs, lactate, and glycerol.
Figure 5: Circadian rhythm genes regulate liver glucose and ketone production from fatty acids, amino acids, glycerol, and other substrates. Ketogenesis generates ~34 ATP, far more than the 2–4 ATP from glucose, helping stabilize blood sugar, fat stores, and improve insulin and leptin sensitivity. It reduces the brain’s reliance on carbs and proteins, easing hunger, mood issues (like anxiety and fatigue), and inflammation from oxidative stress.
Regulation of Cholesterol Metabolism
Cholesterol metabolism in the brain and body is independently regulated, separated by the blood-brain barrier, with the brain relying on liver-synthesized cholesterol. Glucagon activates lipoprotein lipase (LPL) to release free fatty acids (FFAs) for energy or storage. The exogenous pathway packages dietary fats into chylomicrons for liver processing, while the endogenous pathway converts VLDL cholesterol into LDL through triglyceride metabolism, with lipoprotein density decreasing as fat content rises.
Figure 6: The exogenous pathway converts dietary triglycerides into chylomicrons, which deliver nutrients to tissues and shrink as triglycerides are used for energy or storage. Cholesterol esters in the remnants bind to liver receptors for clearance, supporting VLDL cholesterol and bile acid production in the endogenous pathway.
In the small intestine, fatty acids mainly come from dietary fat, while cholesterol is from bile salts (800–1200 mg vs. 200–500 mg from diet). Higher fat intake produces larger chylomicrons (CM), increasing VLDL and LDL levels. During fasting, fatty acids fuel energy, shrinking CM, VLDL, IDL, and LDL. After meals, VLDL triglycerides are 44% plasma FFAs, 10% diet, 15% chylomicron remnants, and 8% lipogenesis; fasting relies 77% on plasma FFAs and 4% on lipogenesis.
Figure 7: Cholesterol is synthesized from acetyl CoA through enzyme-driven reactions and is used to produce steroid hormones, bile salts, vitamin D, oxysterols, and lipoproteins, while also being a key component of cell membranes.
Role of Cholesterol in the Body
Cholesterol maintains cell structure, fluidity, signaling, and communication. It supports skin, hair, temperature regulation, and organ protection. At low temperatures, it prevents lipid packing, while at high temperatures, it reduces membrane fluidity. Cholesterol also synthesizes steroid hormones, vitamin D, bile acids, and aids lipid and fat-soluble vitamin digestion for energy (ATP) production.
Cholesterol is an Integral Part of Cell Membranes
Figure 8: The cell membrane, a phospholipid bilayer, has polar heads outward, nonpolar tails inward, and embedded proteins and carbohydrates. Small nonpolar molecules pass easily, while large polar ones require protein transporters. Cholesterol and tail saturation regulate fluidity, vital for movement and stress response.
Cholesterol is an Integral Part of Synthesizing All Steroid Hormones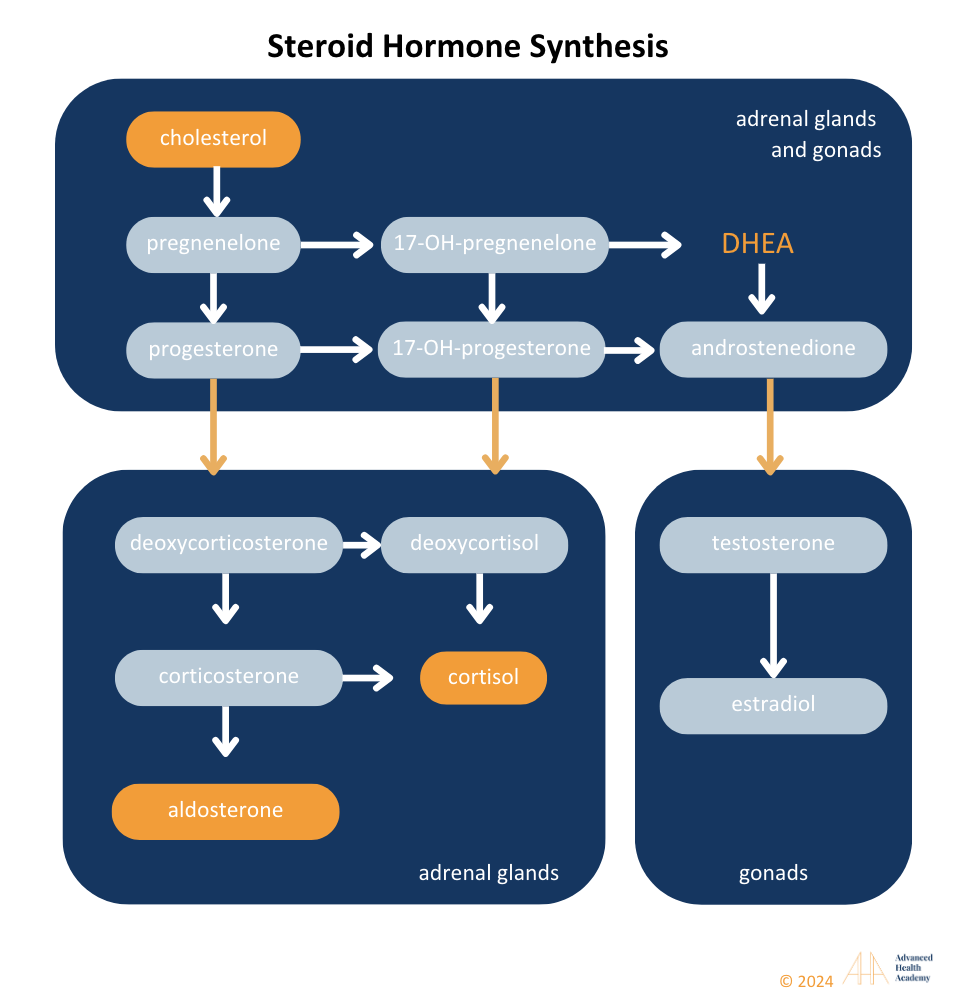
Figure 9: Liver cytochrome P450 enzymes convert cholesterol into steroid hormones, including aldosterone, cortisol, androgens (testosterone, progesterone, estradiol), and DHEA. Defects in this process can disrupt hormones, causing conditions like PCOS or hypogonadism. High cholesterol can increase aldosterone, cortisol, and estradiol while lowering DHEA and testosterone.
Cholesterol is the Precursor for Bile Salt Production
Figure 10: Cytochrome P450 enzymes in the liver convert cholesterol derivatives into bile salts, conjugate bilirubin, and synthesize acids like cholic and chenodeoxycholic acid. Bile is stored in the gallbladder or released into the intestine, where bacterial enzymes deconjugate it to deoxycholic and lithocholic acids.
Bile acids are 80% organic, with the rest being cholesterol and phospholipids. Bile salts aid in digesting and transporting dietary fats, oils, and fat-soluble vitamins (A, D, E, K) to tissues. They also remove excess cholesterol, protecting cells from oxidative stress.
Cholesterol is the Precursor for vitamin D Production
Vitamin D is an essential micronutrient with hormone-like functions. There are two major dietary forms of vitamin D:
vitamin D2 (ergocalciferol) is produced by plants and yeast
vitamin D3 (cholecalciferol) is produced by animals
Figure 11: Cholesterol is used to synthesize vitamin D3. Cholesterol is converted to 7-dehydrocholesterol in the skin and then to vitamin D3 with UV-B sunlight. D3 binds to vitamin D-binding protein or chylomicrons and travels to the liver, where 25-hydroxylase forms 25-hydroxy vitamin D [25(OH)D], the primary marker for vitamin D status.
Cholesterol is an Antioxidant
Figure 12: Oxidation of cholesterol to oxysterols activates Liver X receptors (LXRs), facilitating excess LDL removal from cells and tissues to the liver and spleen for destruction. Oxysterols also aid steroid hormone synthesis. Low oxysterol levels trigger SCAP to transport SREBPs, increasing LDL synthesis. Excess oxysterols inhibit SCAP, reducing cholesterol synthesis, and are stored in bile or excreted via urine or stool .
Oxysterols act as antioxidants, protecting tissues from oxidative stress. When toxins like bacterial byproducts accumulate, oxysterols help store them in fat, shielding organs. Toxins are later expelled via stool, urine, sweat, sebum, bloodletting, or hair growth.
Clinical Significance of Monitoring Total Cholesterol Levels5
Figure 13: Extremely high or low cholesterol can cause multi-organ damage (brain, liver, kidneys, bones, joints, glands) leading to hormonal imbalances, infections, cancer, osteoporosis, osteoarthritis, diabetes, and vascular diseases.
Hyperlipidemia, or high blood lipid levels, often results from unhealthy lifestyle choices, leading to excess fat storage and poor metabolism. It is linked to high triglycerides (TG), VLDL, LDL, and low HDL levels. Low cholesterol, if paired with low TG, VLDL, and LDL and normal or high HDL, is usually not a concern and supports balanced hormone production. Abnormal lipid levels often stem from dysbiosis and reduced blood flow to organs. Gut microbiota play a key role in digestion, nutrient absorption, and waste removal, while delayed stomach emptying can disrupt gut bacteria and cause nutrient deficiencies .
Figure 14: Healthy gut microbiota maintains energy, hormonal, and metabolic balance, aiding digestion, nutrient absorption, and waste removal. Dysbiosis, a loss of healthy bacteria, disrupts metabolism, promotes pathogens, cancer cells, and foreign invaders, and alters immune responses.
Dysbiosis disrupts metabolism, protein and vitamin synthesis, and increases undigested particles like proinflammatory proteins and LDL, leading to hypoxia, liver fat production, and fat storage in VLDL, organs, and bile . Chronic dysbiosis raises ROS levels, causing cell damage and gene mutations. WBCs focus on clearing waste and repairing damage, producing autoantibodies and neglecting pathogens. Oxidative stress-induced atherosclerosis forms plaques and clots, increasing risks for infections, cancer, autoimmune disorders, and organ damage. Dyslipidemia further raises the risk of severe health outcomes.
Figure 15: Dyslipidemia raises the risk of vascular diseases, autoimmune disorders, infections, cancer, and organ damage. While genetics play a role, key causes include dehydration, poor diet, drugs (alcohol, tobacco, medications), and chronic stress.
The consequences of dyslipidemia include, but are not limited to the following diseases:
Liver Dysfunction and Damage
Figure 16: The liver metabolizes cholesterol and proteins, synthesizes apoproteins and amino acids, and detoxifies waste. Liver dysfunction causes excess proinflammatory cholesterol and proteins, depriving organs of nutrients and oxygen. Chronic inflammation (dyslipidemia) leads to liver damage, including steatohepatitis, fibrosis, and cirrhosis.
Dysregulated Hormonal and Energy Balance and Atherosclerosis-Induced Vascular Diseases
A high-cholesterol diet increases LDL receptor expression in liver cells, clearing LDL from the bloodstream . It also boosts luteinizing hormone (LH), follicle-stimulating hormone (FSH), and thyroid-stimulating hormone (TSH) production . LH stimulates androgen production in ovaries, FSH supports follicle development and estrogen synthesis, and TSH promotes thyroid hormones (T3 and T4), enhancing energy production (hyperthyroidism) and reactive oxygen species (ROS).
Excess androgens (e.g., in PCOS) or long-term hormone therapy increase cholesterol synthesis, lower HDL, and promote atherosclerosis by embedding LDL in arteries, forming foam cells, and triggering inflammation . This process reduces fat metabolism, raises LDL, glucose, and insulin, and shifts fat storage to less efficient visceral regions . A 5–10% weight loss improves insulin sensitivity, PCOS, and hyperlipidemia .
Figure 17: Atherosclerosis causes tunica intima thickening, excess clotting, restricted blood flow, high blood pressure, and blood backup in organs like the liver, heart, and pancreas. It overactivates white blood cells and platelets, forming clots and scar tissue that damage cells and disrupt metabolism .
Hyperthyroidism and short-term use of synthetic thyroid hormones (in those with hypothyroidism) can increase fat metabolism, energy harvesting, and HDL cholesterol synthesis. However, long-term hormone replacement therapy and thyroid dysfunction can have the opposite effect: excess conversion of dietary carbohydrates to stored fat downregulates HDL cholesterol synthesis , thereby increasing the concentration of total cholesterol and TG-rich VLDL and LDL particles in the circulation. A Chinese study found high cholesterol (>200 or >240 mg/dL) significantly raises hypothyroidism risk. Statins can improve subclinical hypothyroidism remission rates (50% vs. 15%) .
High-cholesterol diets from animal sources can reduce enzymes like StAR, P450scc, and 3β-HSD, lowering testosterone synthesis.Excess 25-hydroxycholesterol in testicles inhibits Leydig cells’ response to LH, further reducing testosterone . Peptides like Angiotensin 3 and 4 also hinder testosterone production. In contrast, a plant-rich diet lowers cholesterol and improves steroid pathways, increasing testosterone levels.
Autoimmune Disorders
Autoimmune diseases like RA, SLE, IBD, ankylosing spondylitis, Sjögren’s syndrome, polymyalgia rheumatica, periodontal disease, multiple sclerosis, and HIV/AIDS are linked to atherosclerosis-induced vascular inflammation . A meta-analysis of 111,758 patients found RA increased vascular death risk by 50%, with cardiovascular risks similar to diabetes . Lipid abnormalities are common in autoimmune conditions137:
Decrease in HDL-cholesterol and apolipoprotein A-I.
Elevated serum triglyceride and lipoprotein (a) levels.
Decreasing total cholesterol and LDL-cholesterol is associated with severe autoimmune cases.
Chronic Kidney Dysfunction and Damage
High-cholesterol diets lower HDL and increase kidney atherogenic cholesterol deposits, raising CKD risk 55. A 2020 Zhejiang study linked high triglycerides, total cholesterol, and LDL to eGFR decline and CKD incidence due to free fatty acids and reactive oxygen species causing kidney inflammation and scarring . Lowering cholesterol with statins slows eGFR decline. A plant-based, nut, and oil-free diet also benefits CKD patients by reducing further kidney damage.
Skin Disorders
Excess cholesterol on the skin triggers sebum production, disrupting microbiota and allowing bacteria like P. acnes to cause comedones and inflammatory disorders like acne, psoriasis, and xanthomas . Acne is linked to low HDL, high cholesterol, and androgen levels, raising risks for diabetes, gonad dysfunction, and other hyperlipidemia-related conditions. Hyperandrogenism worsens acne, causes hirsutism, and accelerates hair loss (alopecia). The skin uses cholesterol to produce glucocorticoids, estrogen, and DHT. Excess DHT and oxysterols impair scalp hair growth and increase hair loss 128 .
Infections
Cholesterol is vital for cell membranes, but excess atherogenic cholesterol damages cells and promotes pathogen binding, leading to chronic inflammation . Severe infections lower total cholesterol, HDL, and apolipoprotein A-I, predicting higher mortality . Infections are linked to gluconeogenesis, reduced ketogenesis, high blood pressure, obesity, and systemic inflammation. The lipid abnormalities for infections typically involves137:
Decrease in HDL-cholesterol and apolipoprotein A-I
Elevated serum triglyceride and lipoprotein (a) levels
Decreasing total and LDL-cholesterol
Lowering LDL and increasing HDL may reduce infection risks, including COVID-19 complications . Severe COVID-19 and other infections lower HDL and LDL due to liver injury and high proinflammatory proteins, leading to hypoxia and reduced energy production.
Cancer Growth and Migration
Many studies have shown that various cancers are associated with excess total, VLDL, LDL, and triglycerides in the circulation that are ingested by tumor cells, thereby enhancing cancer growth and metastasis48. Extremely high and severely low 25-hydroxycholesterol levels are associated with lower survival rates. The following cancers have a direct correlation with dyslipidemia: pancreatic cancer , colon cancer , breast cancer (especially in overweight and obese individuals) , prostate cancer , liver cancer , lung cancer , ovarian cancer , and chronic lymphocytic leukemia (CLL) .
Figure 18: Excess cholesterol oxidation fuels tumor growth and metastasis. Damaged vessels let tumor cells bind to cholesterol, interact with platelets, and form clots. Neutrophils protect tumor cells, aiding vessel formation and blocking natural killer (NK) cells. Inflammatory proteins from these reactions damage red blood cells, promoting infections, tumor growth, and vessel invasion.
Brain and Nerve Damage
The brain contains high cholesterol levels, synthesized locally as lipoproteins cannot cross the blood-brain barrier . Impaired fat metabolism increases ROS, damaging the barrier and brain cells . White blood cells focus on repairs, reducing clearance of proinflammatory proteins like beta-amyloid . Excess protein deposits disrupt neurons, triggering cholesterol synthesis and mitochondrial activity, leading to neuroinflammatory and neurodegenerative disorders :
Learning disabilities
Cerebrovascular dysfunction
Mild cognitive impairment (familial hypercholesterolemia)
Cognitive impairment with impaired blood-brain barrier and neuroinflammation (such as dementia 169 )
Depression, anxiety, and other mental health disorders
Prevalence and Statistics of Abnormal Cholesterol Levels
Hypercholesterolemia raises the risk of vascular diseases, autoimmune disorders, infections, cancers, and organ damage, causing 2.6 million deaths and 29.7 million disability-adjusted life years (DALYs) globally. In 2008, 39% of adults worldwide had high cholesterol (≥5.0 mmol/L), with the highest rates in Europe (54%) and the Americas (48%), where diets are rich in animal protein. Africa (22.6%) and Southeast Asia (29%) had the lowest rates due to plant-based diets. High-income countries showed over 50% prevalence, compared to 25% in low-income countries 51.
A 2018–2020 Global Diagnostics Network study of 461 million lipid results from 17 countries found cholesterol levels peaked in women at 50–59 years and men at 40–49 years 185. Countries like Japan, Australia, Germany, and Switzerland exceeded average cholesterol levels, reflecting regional differences in diet, genetics, and healthcare practices.
Figure 20: From 2018 to 2020, global data from 17 countries showed dyslipidemia is widespread. High total and LDL cholesterol levels were linked to diets high in animal products or food poverty. Addressing social and economic factors is key to reducing hyperlipidemia in affected regions.
Conclusion
Managing dyslipidemia involves lifestyle changes like a plant-based diet, exercise, intermittent fasting, stress management, avoiding tobacco and alcohol, addressing medication side effects, and maintaining a healthy weight. Medication may help temporarily. Beyond lipid profiles, monitoring apoproteins, lipoprotein size, and concentration can better assess risks for metabolic, inflammatory, and neurological complications. Family history, age, gender, and other health factors are also crucial in determining treatment and promoting overall health.
Source References and Supplemental Research:
- Feingold KR, Anawalt B, Blackman MR, et al. Introduction to Lipids and Lipoproteins. MDText.com, Inc.; 2000. www.endotext.org
- Chait A, Ginsberg HN, Vaisar T, Heinecke JW, Goldberg IJ, Bornfeldt KE. Remnants of the Triglyceride-Rich Lipoproteins, Diabetes, and Cardiovascular Disease. Diabetes 2020; 69:508-516 [PMC free article] [PubMed]
- Krauss RM, King SM. Remnant lipoprotein particles and cardiovascular disease risk. Best Pract Res Clin Endocrinol Metab 2023; 37:101682 [PubMed]
- Berneis KK, Krauss RM. Metabolic origins and clinical significance of LDL heterogeneity. J Lipid Res 2002; 43:1363-1379 [PubMed]
- Asztalos BF, Niisuke K, Horvath KV. High-density lipoprotein: our elusive friend. Curr Opin Lipidol 2019; 30:314-319 [PubMed]
- Thakkar H, Vincent V, Sen A, Singh A, Roy A. Changing Perspectives on HDL: From Simple Quantity Measurements to Functional Quality Assessment. J Lipids 2021; 2021:5585521 [PMC free article] [PubMed]
- Thomas SR, Zhang Y, Rye KA. The pleiotropic effects of high-density lipoproteins and apolipoprotein A-I. Best Pract Res Clin Endocrinol Metab 2023; 37:101689 [PubMed]
- Julve J, Martin-Campos JM, Escola-Gil JC, Blanco-Vaca F. Chylomicrons: Advances in biology, pathology, laboratory testing, and therapeutics. Clin Chim Acta 2016; 455:134-148 [PubMed]
- Daoud E, Scheede-Bergdahl C, Bergdahl A. Effects of Dietary Macronutrients on Plasma Lipid Levels and the Consequence for Cardiovascular Disease. Journal of Cardiovascular Development and Disease. 2014; 1:201-213. 10.3390/jcdd1030201.
- Gerber PA, Nikolic D, Rizzo M. Small, dense LDL: an update. Curr Opin Cardiol. 2017 Jul;32(4):454-459. doi: 10.1097/HCO.0000000000000410. PMID: 28426445.
- Thomas A. B. Sanders (2016): “The Role of Fats in Human Diet.” Pages 1-20 of Functional Dietary Lipids. Woodhead/Elsevier, 332 pages. ISBN 978-1-78242-247-1doi:10.1016/B978-1-78242-247-1.00001-6
- Entry for “fat” Archived 2020-07-25 at the Wayback Machine in the online Merriam-Webster dictionary, sense 3.2. Accessed on 2020-08-09
- “Introduction to Energy Storage”. Khan Academy.
- Wu, Yang; Zhang, Aijun; Hamilton, Dale J.; Deng, Tuo (2017). “Epicardial Fat in the Maintenance of Cardiovascular Health.” Methodist DeBakey Cardiovascular Journal. 13 (1): 20–24. doi:10.14797/mdcj-13-1-20. ISSN 1947-6094. PMC 5385790. PMID 28413578.
- Feingold KR, Grunfeld C. Lipids: a key player in the battle between the host and microorganisms. J Lipid Res 2012; 53:2487-2489 [PMC free article] [PubMed]
- White, Hayden; Venkatesh, Balasubramanian (2011). “Clinical review: Ketones and brain injury.” Critical Care. 15 (2): 219. doi:10.1186/cc10020. PMC 3219306. PMID 21489321
- Abumrad NA, Davidson NO. Role of the gut in lipid homeostasis. Physiol Rev 2012; 92:1061-1085 [PMC free article] [PubMed]
- Olivecrona G. Role of lipoprotein lipase in lipid metabolism. Curr Opin Lipidol 2016; 27:233-241 [PubMed]
- Dallinga-Thie GM, Franssen R, Mooij HL, Visser ME, Hassing HC, Peelman F, Kastelein JJ, Peterfy M, Nieuwdorp M. The metabolism of triglyceride-rich lipoproteins revisited: new players, new insight. Atherosclerosis 2010; 211:1-8 [PMC free article] [PubMed]
- M. Adiels, S.O. Olofsson, M.R. Taskinen, et al., Overproduction of very low-density lipoproteins is the hallmark of dyslipidemia in the metabolic syndrome, Arteriosclerosis, Thrombosis, Vasc. Biol. 28 (2008) 1225e1236.
- Hooper AJ, Burnett JR, Watts GF. Contemporary aspects of the biology and therapeutic regulation of the microsomal triglyceride transfer protein. Circ Res 2015; 116:193-205 [PubMed] [Reference list]
- S. Tiwari, S.A. Siddiqi, Intracellular trafficking and secretion of VLDL, Arteriosclerosis, Thrombosis, Vasc. Biol. 32 (2012) 1079e1086. DOI: 10.1161/ATVBAHA.111.241471
- Daoud E, Scheede-Bergdahl C, Bergdahl A. Effects of Dietary Macronutrients on Plasma Lipid Levels and the Consequence for Cardiovascular Disease. Journal of Cardiovascular Development and Disease. 2014; 1:201-213. 10.3390/jcdd1030201.
- Espenshade, P.J. SREBPs: sterol-regulated transcription factors. J. Cell Sci. 2006, 119, 973-976.
- Dawidowicz, E.A. Dynamics of membrane lipid metabolism and turnover. Ann. Rev. Biochem. 1987, 56, 43–61.
- Wang, H.H.; Garruti, G.; Liu, M.; Portincasa, P.; Wang, D.Q.-H. Cholesterol and Lipoprotein Metabolism and Atherosclerosis: Recent Advances in Reverse Cholesterol Transport. Ann. Hepatol. 2017, 16 (Suppl. S1), S27–S42.
- Sacks, F.M.; Lichtenstein, A.H.; Wu, J.H.; Appel, L.J.; Creager, M.A.; Kris-Etherton, P.M.; Miller, M.; Rimm, E.B.; Rudel, L.L.; Robinson, J.G. and Stone, N.J. Dietary Fats and Cardiovascular Disease: A Presidential Advisory From the American Heart Association. Circulation 2017, 136, e1–e23.
- Di Ciaula, A.; Garruti, G.; Baccetto, R.L.; Molina-Molina, E.; Bonfrate, L.; Portincasa, P.; Wang, D.Q.. Bile acid physiology. Ann. Hepatol. 2017, 16, s4–s14.
- Cox, R.A.; García-Palmieri, M.R. Cholesterol, Triglycerides, and Associated Lipoproteins. In Clinical Methods: The History, Physical, and Laboratory Examinations; Walker, H.K., Hall, W.D., Hurst, J.W., Eds.; Butterworths: Boston, MA, USA, 2011.
- Piskin E, Cianciosi D, Gulec S, Tomas M, Capanoglu E. Iron Absorption: Factors, Limitations, and Improvement Methods. ACS Omega. 2022 Jun 21; 7(24): 20441–20456. doi: 10.1021/acsomega.2c01833
- Benkhedda K.; L’abbé M. R.; Cockell K. A. Effect of Calcium on Iron Absorption in Women with Marginal Iron Status. Br. J. Nutr. 2010, 103 (5), 742–748. 10.1017/S0007114509992418.
- Ziegler E.E. Consumption of cow’s milk as a cause of iron deficiency in infants and toddlers. Nutr. Rev. 2011;69:37–42. doi: 10.1111/j.1753-4887.2011.00431.x.
- Bondi S.A., Lieuw K. Excessive Cow’s Milk Consumption and Iron Deficiency in Toddlers, Two Unusual Presentations and Review. ICAN Infant Child Adolesc. Nutr. 2009;1:133–139. doi: 10.1177/1941406409335481. [CrossRef]
- Gifford GE, Duckworth DH. Introduction to TNF and related lymphokines. Biotherapy. 1991;3:103–111
- De Chiara, F., Checcllo, C. U., & Azcón, J. R. (2019). High protein diet and metabolic plasticity in Non-Alcoholic Fatty liver Disease: Myths and Truths. Nutrients, 11(12), 2985. https://doi.org/10.3390/nu11122985 [PubMed]
- Esse, R.; Barroso, M.; Almeida, I.; Castro, R. The contribution of homocysteine metabolism disruption to endothelial dysfunction: State-of-the-art. Int. J. Mol. Sci. 2019, 20, 867. [Google Scholar] [CrossRef] [Green Version].
- Kruman, I.I.; Culmsee, C.; Chan, S.L.; Kruman, Y.; Guo, Z.; Penix, L.; Mattson, M.P. Homocysteine elicits a DNA damage response in neurons that promotes apoptosis and hypersensitivity to excitotoxicity. J. Neurosci. 2000, 20, 6920–6926.
- Guoyao Wu; Yun-Zhong Fang; Sheng Yang; Joanne R. Lupton; Nancy D. Turner (2004). “Glutathione Metabolism and its Implications for Health”. Journal of Nutrition. 134 (3): 489–492. doi:10.1093/jn/134.3.489. PMID 14988435 [PubMed]
- Pompella A, Visvikis A, Paolicchi A, De Tata V, Casini AF (October 2003). “The changing faces of glutathione, a cellular protagonist”. Biochemical Pharmacology. 66 (8): 1499–1503. doi:10.1016/S0006-2952(03)00504-5. PMID 14555227
- S. Tiwari, S.A. Siddiqi, Intracellular trafficking and secretion of VLDL, Arteriosclerosis, Thrombosis, Vasc. Biol. 32 (2012) 1079e1086. DOI: 10.1161/ATVBAHA.111.241471
- Reichert CO, Levy D, Bydlowski SP. Paraoxonase Role in Human Neurodegenerative Diseases. Antioxidants (Basel). 2020 Dec 24;10(1):11. doi: 10.3390/antiox10010011. PMID: 33374313; PMCID: PMC7824310.
- Sengupta, S.; Chen, H.; Togawa, T.; DiBello, P.M.; Majors, A.K.; Büdy, B.; Ketterer, M.E.; Jacobsen, D.W. Albumin thiolate anion is an intermediate in the formation of albumin-S-S-homocysteine. J. Biol Chem. 2001, 276, 30111–30117.
- Diamond, J.R. Analogous Pathobiologic Mechanism in Glomerulosclerosis and Atherosclerosis. Kidney Int. 1991, 31, 29–34.
- Chen, P.; Poddar, R.; Tipa, E.V.; Dibello, P.M.; Moraveca, C.D.; Robinson, K.; Green, R.; Kruger, W.D.; Garrow, T.A.; Jacobsen, D.W. Homocysteine Metabolism in Cardiovascular Cells and Tissues: Implication for Hyperhomocysteinemia and Cardiovascular Disease. Adv. Enzym. Regul. 1999, 39, 93–109.
- Ho, P.I.; Ashline, D.; Dhitavat, S.; Ortiz, D.; Collins, S.C.; Shea, T.B.; Rogers, E. Folate deprivation induces neurodegeneration: Roles of oxidative stress and increased homocysteine. Neurobiol. Dis. 2003, 14, 32–42.
- M.B. Fessler, The challenges and promise of targeting the Liver X Receptors for treatment of inflammatory disease, Pharmacol. Ther. (2017), https:// doi.org/10.1016/j.pharmthera.2017.07.010 pii: S0163-7258(17)30190-0.
- S.D. Lee, P. Tontonoz, Liver X receptors at the intersection of lipid metabolism and atherogenesis, Atherosclerosis 242 (2015) 29e36.
- D.S. Green, H.A. Young, J.C. Valencia, Current prospects of type II interferon gamma signaling and autoimmunity, J. Biol. Chem. 292 (2017) 13925e13933.
- M. Buttet, H. Poirier, V. Traynard, et al., Deregulated lipid sensing by intestinal CD36 in diet-induced hyperinsulinemic obese mouse model, PLoS One 11 (2016), e0145626.
- Main, P.A.; Angley, M.T.; O’Doherty, C.E.; Thomas, P.; Fenech, M. The potential role of the antioxidant and detoxification properties of glutathione in autism spectrum disorders: A systematic review and meta-analysis. Nutr. Metab. 2012, 9, 35. [Google Scholar] [CrossRef] [PubMed] [Green Version]
- Desai, A.; Sequeira, J.M.; Quadros, E.V. The metabolic basis for developmental disorders due to defective folate transport. Biochimie 2016, 126, 31–42. [Google Scholar] [CrossRef]
- Ali, A.; Waly, M.I.; Al-Farsi, Y.M.; Essa, M.M.; Al-Sharbati, M.M.; Deth, R.C. Hyperhomocysteinemia among Omani autistic children: A case-control study. Acta Biochim. Pol. 2011, 58, 547–551. [Google Scholar] [CrossRef]
- Al-Farsi, Y.M.; Waly, M.I.; Deth, R.C.; Al-Sharbati, M.M.; Al-Shafaee, M.; Al-Farsi, O.; Al-Khaduri, M.M. Low folate and vitamin B12 nourishment is common in Omani children with newly diagnosed autism. Nutrition 2013, 29, 537–541. [Google Scholar] [CrossRef]
- Fuentes-Albero, M.; Cauli, O. Homocysteine Levels in Autism Spectrum Disorder: A Clinical Update. Endocr. Metab. Immune Disord. Drug Targets 2018, 18, 289–296. [Google Scholar] [CrossRef]
- Altun, H.; Kurutaş, E.B.; Şahin, N.; Güngör, O.; Findikli, E. The Levels of Vitamin D, Vitamin D Receptor, Homocysteine and Complex B Vitamin in Children with Autism Spectrum Disorders. Clin. Psychopharmacol. Neurosci. 2018, 16, 383–390. [Google Scholar] [CrossRef]
- Kałużna-Czaplińska, J.; Żurawicz, E.; Michalska, M.; Rynkowski, J. A focus on homocysteine in autism. Acta Biochim. Pol. 2013, 60, 137–142. [Google Scholar] [CrossRef] [PubMed]
- Mattson, M.P.; Shea, T.B. Folate and homocysteine metabolism in neural plasticity and neurodegenerative disorders. Trends Neurosci. 2003, 26, 137–146. [Google Scholar] [CrossRef]
- Ho, P.I.; Ortiz, D.; Rogers, E.; Shea, T.B. Multiple aspects of homocysteine neurotoxicity: Glutamate excitotoxicity, kinase hyperactivation and DNA damage. J. Neurosci. Res. 2002, 70, 694–702. [Google Scholar] [CrossRef] [PubMed]
- Fulceri, F.; Morelli, M.; Santocchi, E.; Cena, H.; Del Bianco, T.; Narzisi, A.; Calderoni, S.; Muratori, F. Gastrointestinal symptoms and behavioral problems in preschoolers with Autism Spectrum Disorder. Dig. Liver Dis. 2016, 48, 248–254. [Google Scholar] [CrossRef]
- Chauhan, A.; Audhya, T.; Chauhan, V. Brain region-specific glutathione redox imbalance in autism. Neurochem. Res. 2012, 37, 1681–1689. [Google Scholar] [CrossRef]
- Rossignol, D.A.; Frye, R.E. Evidence linking oxidative stress, mitochondrial dysfunction, and inflammation in the brain of individuals with autism. Front. Physiol. 2014, 5, 150. [Google Scholar] [CrossRef] [Green Version]
- Frye, R.E.; Melnyk, S.; Fuchs, G.; Reid, T.; Jernigan, S.; Pavliv, O.; Hubanks, A.; Gaylor, D.W.; Walters, L.; James, S.J. Effectiveness of methylcobalamin and folinic acid treatment on adaptive behavior in children with autistic disorder is related to glutathione redox status. Autism Res. Treat. 2013, 2013, 609705. [Google Scholar] [CrossRef]
- Sun, C.; Zou, M.; Zhao, D.; Xia, W.; Wu, L. Efficacy of Folic Acid Supplementation in Autistic Children Participating in Structured Teaching: An Open-Label Trial. Nutrients 2016, 8, 337. [Google Scholar] [CrossRef] [Green Version]
- Bottiglieri, T. Folate, vitamin B12, and neuropsychiatric disorders. Nutr. Rev. 1996, 54, 382–390. [Google Scholar] [CrossRef] [PubMed]
- Ezzaher, A.; Mouhamed, D.H.; Mechri, A.; Omezzine, A.; Neffati, F.; Douki, W.; Bouslama, A.; Gaha, L.; Najjar, M.F. Hyperhomocysteinemia in Tunisian bipolarI patients. Psychiatry Clin. Neurosci. 2011, 65, 664–671. [Google Scholar] [CrossRef]
- Kim, H.; Lee, K.J. Serum homocysteine levels are correlated with behavioral and psychological symptoms of Alzheimer’s disease. Neuropsychiatr. Dis. Treat. 2014, 10, 1887–1896. [Google Scholar] [CrossRef] [Green Version]
- Altun, H.; Şahin, N.; Belge Kurutaş, E.; Güngör, O. Homocysteine, Pyridoxine, Folate and Vitamin B12 Levels in Children with Attention Deficit Hyperactivity Disorder. Psychiatr. Danub. 2018, 30, 310–316. [Google Scholar] [CrossRef] [PubMed] [Green Version]
- Brunzell J, Rohlfing J. The Effects of Diuretics and Adrenergic-Blocking Agents on Plasma Lipids. West J Med. 1986 Aug; 145(2): 210–218. PMC1306877
- Wolinsky H. The effects of beta-adrenergic blocking agents on blood lipid levels. Clin Cardiol. 1987 Oct;10(10):561-6. Doi: 10.1002/clc.4960101010
- Stocker R, Yamamoto Y, McDonagh AF, Glazer AN, Ames BN. Bilirubin is an antioxidant of possible physiological importance. Science. 1987;235(4792):1043-6.
- Baranano DE, Rao M, Ferris CD, Snyder SH. Biliverdin reductase: a major physiologic cytoprotectant. Proc Natl Acad Sci USA. 2002;99(25):16093-8.
- B.R. Barrows, E.J. Parks, Contributions of different fatty acid sources to very low-density lipoprotein-triacylglycerol in the fasted and fed states, J. Clin. Endocrinol. Metabolism 91 (2006) 1446e1452. DOI: 10.1210/jc.2005-1709